
LIGHT SCATTERING: A MAJOR CHALLENGE FOR BIOMEDICAL OPTICAL IMAGING
Optical imaging forms 2D or 3D images using light. Advantages of optical imaging include molecular contrast, safe, high sensitivity, high resolution, and low cost. Moreover, according to the left figure, light wavelength is among the three low-attenuation windows for biomedical imaging [1] (Figure adopted from here.)
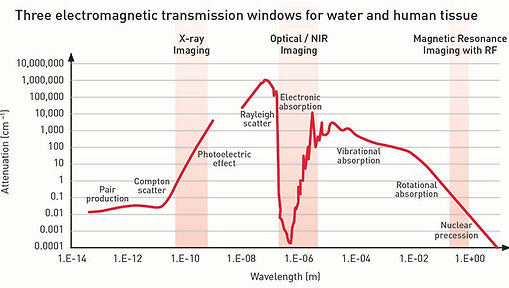

Athough light absorption is weak, the effective penetration of visible and near infrared photons into biological tissue is shallow. This is because at a depth of about 1 mm, photons are on average scattered 10 times and their direction completely randomized [2]. As a result, 1 mm is referred to as the "diffusion limit", which dictates the working depth of almost all optical imaging modalities. As one can see from the right figure, in a clear medium such as water, light follows straight (or at least predictable) trajectories; in contrast, in a scattering medium such as milk, light is scattered into a glowing halo. To image deeper inside tissue using light, it is essential to break the diffusion limit.
OUR SOLUTIONS TO THE SCATTERING PROBLEM:
A BRIEF INTRODUCTION
If we treat scattering as a maze for photons, our goal is to figure out the shape of the target (a red heart symbol in the middle) buried in the maze. We use two methods to acheive this goal: wavefront shaping and photoacoustics.
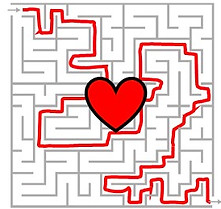
In wavefront shaping [3], we guide photons through the maze to reach the target. Ideally, all the launched photons can interact with the target, and we know exactly where the interaction takes place.
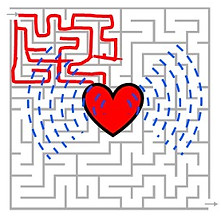
In photoacoustic imaging [4], photons take all possible paths. Some lucky photons interact with the target and convert their energy into sound. We remotely listen to the sound to determine the target location.
OPTICAL TIME REVERSAL:
A FAST WAVEFRONT SHAPING METHOD

There are many ways to do wavefront shaping, a link is provided at the bottom of this page which includes useful information about wavefront shaping techniques. A fast and effective way of performing wavefront shaping is through optical time reversal [5]. Since the wave equation governing the scattering process in a lossless, reciprocal medium exhibits time reversal symmetry, we can reverse the whole scattering process by techniques such as optical phase conjugation. Suppose we introduce a guide star inside the scattering medium at the desired focal position, while external to the medium, we use a time-reversal mirror to reflect photons emitted by the guide star. Those photons will trace back their original trajectories and converge to the guide star location to make a focus.

For biomedical applications, it is essential that the guide star we introduced is non-invasive. Developing effective guide stars is currently under intensive study [6]. In my research, we developed two kinds of non-invasive guide stars: focused ultrasound and time-variant objects. When using ultrasound as a guide star, some of the scattered photons passing through an ultrasonic focus will be frequency shifted.
If we exclusively time-reverse the frequency-shifted photons, they will converge to the ultrasonic focus [7, 8]. When we choose to use time-variant object as the guide star, the perturbation of the scattered light field due to the dynamic object is measured, and a time-reversed differential field is synthesized digitally [9]. Here, subtracting the fields scattered at different times cancels out the static portion contributed by the scattering medium, exposing the field associated with the dynamic object. Using this method, we can focus light onto a moving target (panel a in the above figure), or onto flowing blood (panel b), and even image a time variant object (panel c). Details can be found in our paper published in Nature Photonics here.
PHOTOACOUSTIC IMAGING:
COMBINING OPTICAL CONTRAST AND ULTRASOUND PENETRATION



Although the propagation directions of photons are randomized within the first 1 mm in tissue, light energy can be delivered to much deeper regions (a couple of centimeters) as diffusion waves. Some molecules absorbe preferentially at certain fixed wavelength, giving rise to instant heating and ultrasound emission due to thermal expansion. The intensity of the light-induced ultrasound is molecule-specific, thus we obtain optical absorption contrast. Thanks to the extremely low ultrasound attenuation and aberration, photoacoustic emission at deep regions can be detected, as a result, we obtain optical contrast and ultrasound penetration (many centimeters!).
The figures on the left show cross sections of the mouse body [10]. The upper figure shows the liver region, the lower one shows the kidney. In the images, brighter corresponds to stronger signal. The contrast in these anatomical images mainly comes from blood absorption at 1064 nm. The penetration depth is more than a centimeter. According to the upper panel of the right figure, blood with different oxygen concentration absorbs differently. This allows us to do functional imaging [10] (blood oxygenation, sO2) of the mouse cortex in vivo spectroscopically (bottom right images).

A GLANCE INTO THE FUTURE
Optical imaging is emerging rapidly as a powerful tool for clinical and preclinical applications. However, the shallow penetration of photons due to tissue scattering is a major obstacle to its widespread uses. Our goal is to break through the penetration depth limit imposed by optical scattering and image with optical contrast and high spatial resolution at a depth of many centimeters. We are developing two novel technologies -- optical wavefront shaping and photoacoustic imaging-- to tackle this challenging problem. As these technologies are starting to take off and making extensive impacts, we hope that YOU can join us at this exciting moment and enjoy the fun of deep optical imaging!
REFERENCES
[1] B. W. Pogue, "Optics in the Molecular Imaging Race." Optics and Photonics News 26, 24-31 (2015).
[2] V. Ntziachristos, "Going deeper than microscopy: the optical imaging frontier in biology." Nature Methods 7, 603-614 (2010). (back to text)
[3] Z. Merali, "Optics: Super vision." Nature 518, 158, 2015. (back to text)
[4] L. V. Wang, and S. Hu. "Photoacoustic tomography: in vivo imaging from organelles to organs." Science 335,
1458-1462, 2012. (back to text)
[5] Z. Yaqoob, et al. "Optical phase conjugation for turbidity suppression in biological samples." Nature Photonics
2, 110-115, 2008. (back to text)
[6] R. Horstmeyer, H. Ruan and C. Yang. "Guidestar-assisted wavefront-shaping methods for focusing light into
biological tissue." Nature Photonics 9, 563-571, 2015. (back to text)
[7] X. Xu, H. Liu, and L. V. Wang. "Time-reversed ultrasonically encoded optical focusing into scattering media."
Nature Photonics 5, 154-157, 2011. (back to text)
[8] [Y. Liu, P. Lai], C. Ma, X. Xu, A. Grabar and L. V. Wang, "Optical focusing deep inside dynamic scattering
media with near-infrared time-reversed ultrasonically encoded (TRUE) light." Nature Communications 6, 2015.
[9] C. Ma, X. Xu, Y. Liu and L. V. Wang, "Time-reversed adapted-perturbation (TRAP) optical focusing onto
dynamic objects inside scattering media." Nature Photonics 8, 931-936, 2014. (back to text)
[10] [L. Li, L. Zhu, C. Ma], et al., "Single-impulse Panoramic Photoacoustic Computed Tomography of Small-
animal Wholebody Dynamics at High Spatiotemporal Resolution" , under review. (back to text)
Note: [authors] denotes equal contribution.